
Romi 7.7 serial key or number
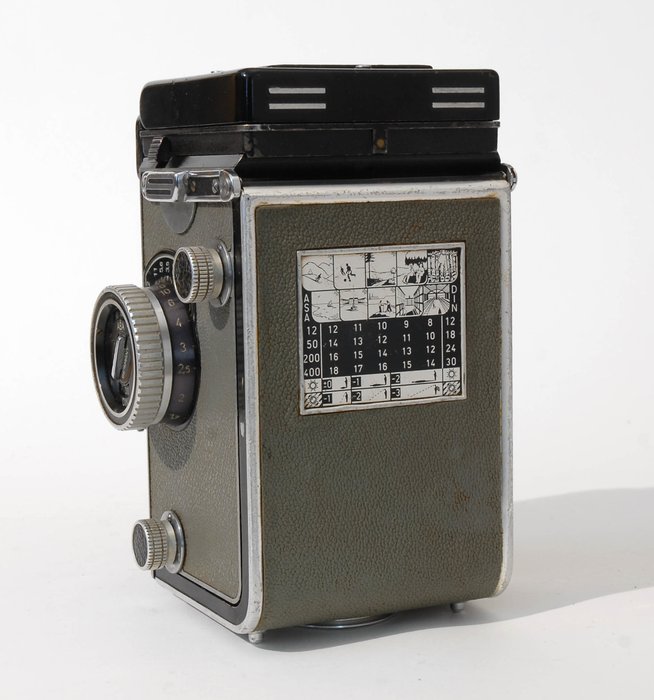
Romi 7.7 serial key or number
PMC
Denise L. Doolan
Queensland Institute of Medical Research, The Bancroft Centre, 300 Herston Road, Post Office Royal Brisbane Hospital, Brisbane, Queensland 4029, Australia,1 Barcelona Centre for International Health Research, Hospital Clínic/IDIBAPS, Universitat de Barcelona, Spain,2 Eijkman-Oxford Clinical Research Unit, Jalan Diponegoro No. 69, Jakarta, Indonesia,3 Centre for Tropical Medicine, Nuffield Department of Clinical Medicine, Oxford University, Oxford, United Kingdom4
Carlota Dobaño
Queensland Institute of Medical Research, The Bancroft Centre, 300 Herston Road, Post Office Royal Brisbane Hospital, Brisbane, Queensland 4029, Australia,1 Barcelona Centre for International Health Research, Hospital Clínic/IDIBAPS, Universitat de Barcelona, Spain,2 Eijkman-Oxford Clinical Research Unit, Jalan Diponegoro No. 69, Jakarta, Indonesia,3 Centre for Tropical Medicine, Nuffield Department of Clinical Medicine, Oxford University, Oxford, United Kingdom4
J. Kevin Baird
Queensland Institute of Medical Research, The Bancroft Centre, 300 Herston Road, Post Office Royal Brisbane Hospital, Brisbane, Queensland 4029, Australia,1 Barcelona Centre for International Health Research, Hospital Clínic/IDIBAPS, Universitat de Barcelona, Spain,2 Eijkman-Oxford Clinical Research Unit, Jalan Diponegoro No. 69, Jakarta, Indonesia,3 Centre for Tropical Medicine, Nuffield Department of Clinical Medicine, Oxford University, Oxford, United Kingdom4
Abstract
Naturally acquired immunity to falciparum malaria protects millions of people routinely exposed to Plasmodium falciparum infection from severe disease and death. There is no clear concept about how this protection works. There is no general agreement about the rate of onset of acquired immunity or what constitutes the key determinants of protection; much less is there a consensus regarding the mechanism(s) of protection. This review summarizes what is understood about naturally acquired and experimentally induced immunity against malaria with the help of evolving insights provided by biotechnology and places these insights in the context of historical, clinical, and epidemiological observations. We advocate that naturally acquired immunity should be appreciated as being virtually 100% effective against severe disease and death among heavily exposed adults. Even the immunity that occurs in exposed infants may exceed 90% effectiveness. The induction of an adult-like immune status among high-risk infants in sub-Saharan Africa would greatly diminish disease and death caused by P. falciparum. The mechanism of naturally acquired immunity that occurs among adults living in areas of hyper- to holoendemicity should be understood with a view toward duplicating such protection in infants and young children in areas of endemicity.
INTRODUCTION
Each year malaria infects about one-half billion people, killing 1 million to 2 million and severely dampening economic development (44, 123, 133, 289, 321a, 321b). The parasitic Plasmodium species causing malaria persist and even flourish despite the availability of tools for prevention, control, and treatment. Those tools consist of an array of drugs, diagnostics, and insecticides and a detailed understanding of the breeding site preferences of the many anopheline mosquito vectors. Despite the tremendous strides in biotechnology during the past 5 decades and the application to malaria of the many breakthroughs in molecular biology, genetics, immunology, and vaccinology by talented researchers, useful vaccines of any type evade us. This review examines one factor that may contribute substantially to this failure: inadequate understanding of naturally acquired immunity (NAI).
The dawn of scientific understanding of malaria occurred on 6 November 1880, when Alphonse Laveran observed a male gametocyte exflagellating in a blood smear from an Algerian patient with malaria. This event marked the identification of plasmodia as the cause of malaria (181). Working in India in 1897, Ronald Ross identified plasmodial oocysts in the guts of mosquitoes fed on parasitemic birds, thereby implicating mosquitoes as the vector of malaria (261). William George McCallum confirmed plasmodial exflagellation as a process of sexual reproduction in 1897 (200, 201), and Batistta Grassi et al. confirmed anopheline mosquitoes as the vector of human malaria in 1900 (130).
Human malaria has persisted through the development of miracle drugs and insecticides, a global eradication effort, and 30 years of intensive efforts to develop a practical vaccine. Not only does malaria persist; it thrives. Today the global malaria situation is “serious and becoming worse” according to the WHO. The incidence and range of malaria, which were pushed to lows in about 1965 (the zenith of dichlorodiphenyltrichloroethane spraying campaigns), now increase sharply in areas of endemicity and spread into areas where control or eradication had been achieved. Worse still, this resurgence has been in progress for 40 years. Even as early as 1978 the historian Gordon Harrison wrote of the persistence of malaria in the face of such vigorous efforts to attack it, “Failure so universal, so apparently ineluctable, must be trying to tell us something. The lesson could be of course that we have proved incompetent warriors. It could also be that we have misconstrued the problem”(140). Three dominant factors account for the failure to maintain control: (i) parasite resistance to safe and affordable antimalarials, (ii) the almost complete demise of vector control programs in developing tropical and subtropical countries, and (iii) the failure to develop a practical vaccine that prevents malaria. Inadequate understanding of the mechanisms of naturally acquired clinical immunity against plasmodia may be an important factor contributing to the failure to develop a practical vaccine. We explore this possibility by examining the genesis and character of the current state of understanding of NAI.
In 1980, Bruce-Chwatt (50) wrote, “Malaria immunity may be defined as the state of resistance to the infection brought about by all those processes which are involved in destroying the plasmodia or by limiting their multiplication. Natural (innate) immunity to malaria is an inherent property of the host, a refractory state or an immediate inhibitory response to the introduction of the parasite, not dependent on any previous infection with it. Acquired immunity may be either active or passive. Active (acquired) immunity is an enhancement of the defense mechanism of the host as a result of a previous encounter with the pathogen (or parts thereof). Passive (acquired) immunity is conferred by the prenatal or postnatal transfer of protective substances from mother to child or by the injection of such substances.”
In humans, various types of acquired or adaptive immunity against plasmodia have been defined: (i) antidisease immunity, conferring protection against clinical disease, which affects the risk and extent of morbidity associated with a given parasite density; (ii) antiparasite immunity, conferring protection against parasitemia, which affects the density of parasites; and (iii) premunition, providing protection against new infections by maintaining a low-grade and generally asymptomatic parasitemia (171-174, 276). Here, protection is defined as objective evidence of a lower risk of clinical disease, as indicated by both the absence of fever (axillary temperature of >37.5°C) with parasitemia and lower densities of parasitemia.
Across sub-Saharan Africa where the disease is holoendemic, most people are almost continuously infected by P. falciparum, and the majority of infected adults rarely experience overt disease. They go about their daily routines of school, work, and household chores feeling essentially healthy despite a population of parasites in their blood that would almost universally prove lethal to a malaria-naive visitor. This vigor in the face of infection is NAI to falciparum malaria. Adults have NAI, but infants and young children, at least occasionally, do not. NAI is compromised in pregnant women, especially primigravidae, and adults removed from their routine infections apparently lose NAI, at least temporarily. Interventions that reduce exposure below a level capable of maintaining NAI risk the possibility of catastrophic rebound, as occurred in the highlands of Madagascar in the 1980s, with epidemic malaria killing more than 40,000 people (259). Routine exposure to hyper- to holoendemic malaria protects a majority of individuals while killing a minority. Aggressive interventions that consider only that vulnerable minority risk compromising or eliminating the solid protection against severe malaria in the majority.
This review summarizes what is understood about naturally acquired and experimentally induced immunity against malaria, with the help of evolving insights provided by biotechnology, and places these insights in the context of historical, clinical, and epidemiological observations. Apart from the practical importance of understanding NAI with respect to attacking holoendemic malaria, we also undertake this task to emphasize that NAI may be a good model for vaccine development. Consider a vaccine that allows infants and young children the same immunity enjoyed by their older siblings and parents: no disease with natural boosting, lifelong. Even if that concept were to be rendered superfluous by a safe eradication strategy, a more thorough understanding of NAI would almost certainly arm vaccinologists with other concepts to explore and adapt to specific populations. The exploration of NAI is key to the rational development and deployment of vaccines and other malaria control tools for almost any population at risk and, ultimately, a necessary foundation upon which to develop strategies of eradication by any means.
LIFE CYCLE AND GEOGRAPHIC DISTRIBUTION
Natural transmission of malaria occurs through exposure of a human host to the bite of an infective female anopheline mosquito. It is estimated that mosquitoes generally transmit fewer than 100 sporozoites per bite (241, 260, 306, 307). The traditional view has been that a feeding mosquito inoculates sporozoites from its salivary glands into the peripheral circulation of the host, which results, ultimately, in the invasion of hepatocytes. However, recent studies using intravital imaging have shown that sporozoites are injected by mosquitoes into the skin, where they can remain for up to 6 h (323), and that approximately one-third of those leaving the injection site may enter lymphatics and drain to the regional lymph nodes (7); other sporozoites trickle into the bloodstream and traffic to the liver, resulting in multiple potential sites for sporozoite-host interaction. In the P. berghei model, up to 80% of sporozoites injected by mosquito bite were estimated to infect the liver (122). Despite these relatively high numbers of sporozoites that leave the skin and move to the liver, the capacity of each of these sporozoites to result in asexual erythrocytic-stage infections is low. In humans, it takes the bites of five P. falciparum-infected mosquitoes to ensure that 100% of volunteers become infected (249, 310). In the P. yoelii rodent malaria model system, in which one can frequently infect 100% of inbred mice by intravenous administration of 20 sporozoites (the 50% infectious dose for BALB/c, C57BL/6, A/J, and B10.BR mice has been determined to be 4.9 to 10.6 sporozoites [32]), it takes the bites of six to eight infected mosquitoes to achieve 100% infection. This indicates that the majority of sporozoites inoculated by mosquitoes do not lead to productive infections.
It is now well established that Plasmodium sporozoites migrate through Kupffer cells and several hepatocytes before finally infecting a hepatocyte (157, 222). In hepatocytes, the uninucleate sporozoites undergo a cycle of asexual amplification called schizogony that lasts 2 to 10 days, depending on the species (a minimum of 5.5 days among plasmodia infecting humans), with each exoerythrocytic schizont containing as many as 30,000 uninucleate merozoites. Clinical symptoms of malaria are not manifest during liver-stage maturation. In P. vivax infection, sporozoites can remain within hepatocytes in dormant stages known as hypnozoites that can cause clinical relapses (177). Exoerythrocytic schizonts rupture, and the merozoites are then released into the bloodstream where they quickly invade red blood cells (90), commencing the erythrocytic stage of the disease that is responsible for the clinical symptoms. A recent study with the P. yoelii model shows that liver merozoites are released from hepatocytes as merosomes, clusters of 100 to 200 parasites surrounded by a host cell membrane (10).
The invading merozoite carries with it to the interior of the red blood cell an enveloping membrane derived from the host cell, and within an associated parasitophorous vacuole the parasite commences further asexual development. After maturation from the ring stage (immature trophozoite) to a trophozoite and then to a schizont, the parasite undergoes three to six mitotic divisions to yield 6 to 36 merozoites within each erythrocytic schizont (ranges depend on the species). After 48 h, the schizonts rupture, releasing merozoites into the bloodstream. Some of these invade uninfected red blood cells and repeat the cycle of blood schizongony.
Other parasites differentiate into male or female gametocytes that circulate independently in the peripheral blood. P. falciparum gametocytes appear in peripheral circulation about 7 to 15 days after the initial invasion of erythrocytes, but it remains unclear what factors stimulate gamecytogenesis (105). Without treatment, most patients with falciparum malaria will develop gametocytemia within 10 to 40 days after the onset of parasitemia (80, 82). P. vivax gametocytes, in contrast, appear in peripheral blood before clinical symptoms. If ingested by a feeding anopheline mosquito, these forms differentiate to gametes capable of combining to form a diploid zygote where meiosis occurs. The zygote differentiates to an invasive ookinete that penetrates the gut wall and attaches to the outer aspect of the mosquito gut. Bathed in hemolymph, the ookinete differentiates to an oocyst which balloons in size as schizogony produces many thousands of haploid sporozoites. The mature oocyst ruptures, and the sporozoites migrate actively to the mosquito salivary glands. The sporozoites penetrate the glands and rest in the channels bearing saliva, awaiting access to a vertebrate host.
Four species of plasmodia routinely infect humans: P. falciparum, P. vivax, P. malariae, and P. ovale. The greatest impact on human health in terms of mortality is from P. falciparum. The pan-tropical distribution of the parasite, its potentially lethal course of infection, and a profile of increasing resistance to chemoprophylactic and chemotherapeutic agents justifiably make this species a primary focus of concern. Nonetheless, P. vivax also constitutes an important burden on public health throughout most of the tropical and many subtropical or temperate latitudes. Although less often fatal, this infection causes a severely debilitating disease with frequent and sometime multiple episodes of relapse. Moreover, recent reports reveal a significant risk of severe disease and death caused by the same spectrum of syndromes typically linked to falciparum malaria (23, 126, 300). Resistance of P. vivax to standard antimalarials apparently emerged relatively recently compared to that of P. falciparum (252), but prophylactic and therapeutic failure of chloroquine against P. vivax now dominates over chloroquine-sensitive infections on the island of New Guinea (17, 22). At one study site in Indonesian New Guinea (Papua, formerly known as Irian Jaya), 95% of patients with slide-proven vivax malaria had ordinarily curative levels of drug when reporting ill to clinics (22). Primaquine, the only available drug capable of preventing relapses of vivax malaria, may routinely fail as a consequence of parasite resistance, tolerance, or very poor effectiveness driven by poor adherence to the 14-day treatment regimen (21). P. malariae also occurs throughout the tropics, but it tends to appear in isolated pockets and at relatively low frequency compared to P. falciparum or P. vivax. Chloroquine-resistant P. malariae has been reported from southern Sumatra, Indonesia (188). Microscopically confirmed P. ovale is exceedingly rare in eastern Indonesia, New Guinea, and the Philippines but is relatively common in West Africa. Little is known about the clinical susceptibility of this parasite to standard antimalarials. The geographical distribution, prevalence, lethality, and drug resistance risk of the human malaria parasites are summarized in Table Table11.
TABLE 1.
Geographical distribution, prevalence, lethality, and drug resistance risk of the human malaria parasites
Species | Range | Prevalence | Lethality risk | Drug resistance risk | Relapse | Animal reservoir |
---|---|---|---|---|---|---|
P. falciparum | Pan-tropical | High | High | High | No | No |
P. vivax | Pan-tropical, temperate | High | High (?) | High | Yes | No |
P. malariae | Tropical | Low, focal | Low | Low | No | No (?) |
P. ovale | West Africa, Southeast Asia | Rare | Low | Low | Yes | No |
P. knowlesi | Southeast Asia | Rare | High (?) | Low | No | Yes |
Recent work by Singh, Cox-Singh, and their colleagues in Malaysian Borneo provides compelling evidence that a fifth species may in fact routinely infect humans. They uncovered evidence of P. knowlesi, which naturally infects macaques on that island (and many other areas of southeast Asia), routinely infecting humans living in proximity to the monkeys, causing acute illness and some deaths (92, 93, 283).
Malaria is not an exclusively tropical disease. Numerous small outbreaks in North America over the past 20 years emphasize the fact that malaria reached well into temperate climates as recently as 100 years ago. Even today malaria in the Koreas and much of temperate Middle East illustrates the ability of these parasites (in particular P. vivax, with its ability to lay dormant in the liver for weeks, months, or years) to thrive under seasonally favorable conditions.
HISTORICAL OBSERVATIONS OF NAI
Knowledge of an acquired protection against malaria predates any information on the specific cause of the disease. European colonists in the tropics long understood their dangerous susceptibility to malaria compared to indigenous people. It was obvious to them that those exposed to malaria since birth enjoyed a very high degree of protection, although many attributed such distinctions to genetic constitution (not entirely erroneously) rather than to acquired immunity. In 1900, Robert Koch first reported a scientific basis for naturally acquired protection against malaria (171-174). Using cross-sectional studies of stained blood films, an innovation at that time, Koch examined the frequency and density of parasitemia in two distinct populations: (i) those in an area of low endemicity at Sukabumi, West Java, and (ii) those in an area of high endemicity at Ambarawa, Central Java. Koch deduced that protection against malaria was acquired only after heavy and uninterrupted exposure to the parasite. The relatively uniform distribution (frequency and density) of parasites across age groups at Sukabumi contrasted with the distinct age-dependent patterns seen at Ambarawa. Koch's report was followed by a number of cross-sectional microscopic studies of malaria in communities in Asia and Africa that largely corroborated the findings on Java. By 1920, the essential features of NAI had been described. It was accepted that natural immunity was (i) effective in adults after uninterrupted lifelong heavy exposure, (ii) lost upon cessation of exposure, (iii) species specific, (iv) somewhat stage specific, and (v) acquired at a rate which was dependent upon the degree of exposure (274, 277). These findings put into context the classic studies of epidemic malaria in India reported by Christophers (64, 65), Gill (127), Byam and Archibald (55), and Covell and Baily (88). Epidemic malaria killed tens of thousands of people of all ages because the level of exposure before the epidemic was not sufficient to induce protective immunity. Studies of the strain specificity of NAI awaited therapeutic challenge with malaria for advanced syphilis in the 2 decades remaining before penicillin appeared to supplant that therapy.
HISTORICAL OBSERVATIONS OF ACTIVELY ACQUIRED OR INDUCED IMMUNITY
The advent of “malariatherapy” of patients suffering from general paresis (neurosyphilis) in 1917 by von Wagner-Jauregg set the stage for conclusively demonstrating that effective immunity against malaria could be induced in humans. Malariatherapy effectively cured about one of three individuals treated in crowded syphilis sanitariums across Europe and the United States, a feat which earned von Wagner-Jauregg the Nobel Prize in 1927. A series of published clinical studies involving thousands of patients largely confirmed conclusions from field work (summarized in references 89, 161, and 208). Although achievable after a single infection, induction of adequate protective immunity usually required repeated infections, and protection against P. falciparum appeared to be acquired more slowly than that against P. vivax or P. malariae. Protective immunity to P. vivax did not persist for a long period of time in the absence of reexposure, as evidenced by the eradication of acute or chronic vivax malaria in previously malaria-naive prisoners and subsequent rechallenge studies with P. vivax sporozoites or trophozoites (326). Immunity was species specific, since immunity to a particular species conferred no protection against challenge with a heterologous species (67, 159, 161, 325). However, it was not necessarily strain specific, since protection could be obtained against challenge with heterologous strains as assessed by shortened clinical episodes and reduced levels of parasitemia, although the protection was not quite as effective as that against the homologous strain (38, 40, 161). Furthermore, it appeared that repeated infections brought about a broadening of specificity, i.e., often transcending the strain (267). A series of retrospective examinations of the malariatherapy studies with P. vivax and P. falciparum were published in 1999 and 2004 (80, 82). The apparently strict species specificity of acquired immunity described in these treatments figures later in the interpretation of observations from Indonesian New Guinea where P. falciparum and P. vivax occur together.
After the historic paper by Brown and Brown (47) demonstrating antigenic variation by P. knowlesi in rhesus macaques, a theory explaining the slow onset of immunity in the field emerged. It was reasoned that repeated infections achieved a sufficiently diverse repertoire of antigenic memory to eventually defeat most variants encountered in the wild. In other words, heavy exposure allowed an accumulation of antigenic memory such that, after 8 to 15 years, there resulted protective albeit nonsterilizing immunity. The relatively rapid onset of protective immunity to malaria in neurosyphilis patients receiving malaria therapy, which was seemingly incompatible with this interpretation, was turned into supporting evidence by pointing to the use of homologous strains in many of those experiments. The often superior protection provided by homologous versus heterologous strains (which was only obvious after the first rechallenge) has been the narrow focus of advocates of cumulative acquisition of NAI. Each episode of infection presumably induced immunity to only that strain. The broader view shows dramatic strain-transcending protection that rapidly eradicates the homologous strain advantage with repeated exposure. Indeed, Brown and Brown (47) themselves pointed to this phenomenon in an obscure and rarely cited section of their paper.
An important pitfall in most of those studies, including the challenge studies using nonhuman primates, was the exclusive use of adult subjects. The extrapolation of findings for adult subjects to a human population that includes susceptible infants and children may well have been a critical error. This issue will be detailed later in this paper.
CHARACTERISTICS OF NAI
The complexity of NAI among age groups increases when the level of exposure falls below those seen in the Sahel of Africa, an area of holoendemicity. Across even just Africa, transmission may be perennial and much less intense, sharply seasonal and as intense, or limited to sporadic epidemics at elevations challenging anopheline tolerances and capacity to serve as a vector. An exploration of the characteristics and determinants of NAI in these far more complex settings is beyond the scope of this review. Our purpose here is to explore the basis of NAI as it occurs in settings of perennial intense transmission.
The principal features of NAI have been defined, but little is known about the underlying mechanisms. The development of clinical and parasitological immunity to malaria is marked by the ability to control disease and parasite density. Parasite density is linked to disease, and diminished parasite counts almost certainly contribute to diminished risk of disease. The dominant factor driving protection from disease may be specific to effectors that diminish parasite numbers, but other effectors, e.g., responses that diminish proinflammatory cytokines, may also play a role. In areas of heavy transmission, the prevalence of parasitemia and the risk of morbidity and mortality caused by malaria decrease markedly with age beyond early childhood. Young children exhibit an “antidisease immunity” which affects the risk and extent of morbidity associated with a given parasite density. The protection seems to be rapidly acquired and results in reduced mortality or severe clinical disease, at least acutely. In contrast, the seemingly slowly acquired “antiparasite immunity” confers protection against high-density parasitemia and the attendant risk of severe disease (192). Sterilizing immunity against infection is never fully achieved, and an asymptomatic carrier status is the rule among adults. This phenomenon of a high degree of immune responsiveness together with the nearly permanent presence of relatively low densities of parasites was originally described by Koch in 1900 and is often termed “premunition” (276). In the absence of continual exposure, the solid immunity against severe disease is apparently relatively short lived. In its usual context, premunition suggests an immunity mediated directly by the presence of the parasites themselves and not as much the result of previous infections. Premunition in helminth infections is a good example; i.e., there is apparent protection from reinfection with resident worms that is immediately lost when the worms are eliminated. In the milieu of holoendemic transmission, it is difficult to sort out which may be the dominant factor of protection seen in older children and adults, i.e., circulating parasites or the immunity to them that persists even in their absence. Passive transfer experiments, described below, demonstrated an acquired and persistent immunity at work, rather than premunition in its usual context.
In naive individuals of any age, P. falciparum infection is almost always symptomatic, and clinical symptoms can be observed even at very low parasitemia levels. The immunity to asexual blood stages where transmission is low or seasonal has not been adequately explored, and this review does not fully describe the complexity of those settings. The persistence of low-grade, asymptomatic parasitemias, as in the Peruvian Amazon, for example (42), may involve mechanisms of immune protection distinct from those occurring in hyper- to holoendemic transmission. This review largely excludes immunity in settings intermediate between exposure of the genuinely malaria-naive and that of the chronically and heavily exposed. While intermediate exposures and states of immunity clearly represent important topics, the focus here aims at a grasp of NAI mechanisms in the perhaps less complex setting of exposure saturation and maximal levels of protection afforded. We consider this a logical starting point and basis for comparison for the exploration of immunity in intermediate-transmission settings.
In areas of heavy endemicity, disease is sometimes distinct from parasitemia, and both are age dependent. Among very heavily exposed children, high-density parasitemia may occur in the absence of overt clinical symptoms. The greatest disease risk for these children is severe anemia rather than cerebral malaria or failure of respiratory, renal, or hepatic systems. Adults in such areas rarely have high-density parasitemia, but when they do, the symptoms appear to be more severe than those in children with equal parasitemia density and where there is higher risk of disease (236). These rare symptomatic adults may represent the small fraction of the adult population who defy the odds of reinfection long enough for their immunity to wane.
The prevalence of parasitemia increases sharply beginning at about 20 weeks of age. Nonetheless, children remain remarkably resistant to high parasitemia, fever, and severe disease until about 6 months of age. This protection has been thought to be associated with the presence of maternal immunoglobulin G (IgG) antibodies, since IgG is acquired by the fetus in utero, mainly during the third trimester of pregnancy, and IgG levels decrease from birth over the first year of life. However, at least one study has ruled out maternal antibodies against malaria antigens as the basis of this protection (254). Alternatively, the protection of infants may be associated with parasite growth-inhibitory factors such as lactoferrin and secretory IgA found in breast milk and in maternal and infant sera (165). At between 4 and 10 months of age many infants are seronegative to specific malaria antigens (1, 254), but the kinetics of decay of maternal antibodies show interindividual variations which depend on the antigen and the epidemiological setting (119).
The risk of clinical disease increases from birth to about 6 months of age, depending on the transmission rate, and beginning at around 3 to 4 months of age, infants become susceptible to severe disease and death. The risk of cerebral malaria increases with age in children 2 to 4 years old. At about 2 to 5 years of age, the frequency of clinical disease begins to diminish and the risk of mortality sharply decreases. The age of onset of this protection is somewhat earlier with heavier transmission, but protection rarely occurs before the age of 2 years. The presence and density of P. falciparum parasitemia at any given time do not correlate well with clinical disease in areas of holoendemicity. Children may have high parasite loads but no symptoms, or they may have disease with low-density parasitemia. Parasite density in the peripheral circulation as measured by microscopic evaluation of blood smears does not always reflect the full parasite load, as mature parasites may be sequestered in deep organs. Longitudinal studies measuring parasitemias in blood daily and autopsy studies quantifying parasites in tissues are valuable to provide a more complete picture of the true parasite load. In addition, low-level submicroscopic parasitemias may be important in the onset and maintenance of immunity/premunition.
After the age of peak parasite prevalence, the number of clinical attacks of malaria per year dramatically declines, as does the risk of mortality. From adolescence onwards, severe disease very rarely occurs. Mild clinical episodes may still be quite common, and the cumulative incidence of parasitemia often approaches 100% within just a few months (19, 236). People having chronic, heavy, and largely uninterrupted exposure to infection develop and maintain a highly efficacious protection from severe disease at an age corresponding roughly with the onset of puberty. Studies by Kurtis and colleagues (178) suggest that the onset of puberty itself, rather than cumulative exposure linked to calendar age, may be a dominant factor in the onset of protective immunity. Intrinsic factors linked to a maturing immune system may be one key to understanding the molecular and cellular bases of NAI.
The characteristics of NAI against malaria substantially change during pregnancy. Despite the effective immunity against severe disease that comes with reaching adulthood in areas of heavy endemicity, pregnant women demonstrate a markedly increased susceptibility to malaria (indicated by increased parasite densities and risk of severe disease and death), particularly during first and second pregnancies (reviewed in reference 212). Significant correlations between host maternal parity and risk of pathogenic infection have been reported, and parasite density decreases as the number of gestations increases (87, 99). Maternal susceptibility to malaria infection during pregnancy is thought to be related to the physiological immunosupression that occurs during gestation (213) and the accumulation of erythrocytes infected with P. falciparum in the placenta through cytoadherence mechanisms (28). The specifics of malaria immunity during pregnancy and the associated pathology have being reviewed recently (29, 124, 256, 257) and will not be considered in detail here; however, host factors that may in part explain the susceptibility of pregnant women to malaria include (i) impairment of cellular immunity, as the concentrations of some cytokines (e.g., tumor necrosis factor alpha) in the placenta have been shown to be significantly higher among primigravidae with severe anemia than among other primigravidae and have been correlated with densities of P. falciparum-infected erythrocytes and of intervillous monocyte infiltrates in the placenta (258), and (ii) hormonal immunosuppression, where a sustained increase in the levels of hormones associated with pregnancy may underlie the increased susceptibility of pregnant women, particularly primigravidae, to malaria. Levels of corticosteroids, which suppress cell-mediated immunity, are substantially increased during the third trimester of pregnancy, in primiparous women, and in P. falciparum-infected pregnant women compared with other pregnant women (311). Cortisol concentrations have been found to be significantly higher in primigravidae than in multigravidae; conversely, plasma prolactin levels have been highest in multigravidae (37). Placental P. falciparum infection is also likely to have an effect on the development of immunity in the offspring due to in utero sensitization to parasite antigens (reviewed in references 45 and 107), and this research area has recently gained increasing interest.
EFFICACY OF NAI
NAI provides solid protection against severe morbidity and mortality. Older children and adults in areas of hyper- to holoendemicity rarely experience life-threatening complications caused by malaria. Even mild disease is relatively uncommon. Quantitative demonstrations of the relative immunity of adults living under conditions of hyper- to holoenemic transmission have corroborated this view. In a study in northern Ghana, an area of holoendemicity, 2% of adults in a wet-season cohort of 192 individuals had a first parasitemia after radical cure that was >20,000 parasites/μl. In that cohort, 97% had a parasitemia during the 16 weeks of follow-up (236). In contrast, 32% of infants and young children (age 6 to 24 months) in a wet-season cohort of 254 had parasite densities of >20,000/μl. Most of the subjects with these high-grade parasitemias were ill, children and adults virtually alike (78 of 81 children with fever, versus 3 of 4 adults). Prevention of high-density parasitemia appears to be the basis of adult protection against disease. The relative risk of wet-season parasitemia exceeding 20,000/μl in children was 21 (95% confidence interval [CI] = 8 to 78; P < 0.00001) relative to adults. In other words the protective efficacy of NAI against high-density parasitemia was 94%. The efficacy of adult protection against death has not been similarly measured, but we believe that it must approach 100%.
The risk of death among young children should also be put into the perspective that includes risk of the same among those who lack continuous chronic exposure to infection. There is no precise estimate of the risk of death with P. falciparum in the absence of immunity or chemotherapy in any age group. However, fatality rates among nonimmune Europeans traveling in areas of holoendemicity in Africa during the 19th century provide some clue to the magnitude of risk in malaria-naive adults. McGregor (207) recounted a European expedition on the Niger River during the middle of the 19th century in which 28% of Caucasians died of fevers presumed to be malaria, despite the likely availability of quinine. Mortality due to malaria among French troops posted in Senegal between 1819 and 1831 was reported to range from 9% to 57% (95). One may thus conservatively estimate 30% as the risk of death without acquired immunity or adequate chemotherapeutic management of malaria. In contrast, an estimated 2% of African children succumb to death caused by malaria before the age of 5 years (131). Thus, it seems that the African children, even those within the ages of highest vulnerability, enjoy quite a large degree of protection from severe disease and death. A study by Gupta et al. suggested that this is the case in areas of intense transmission, showing by mathematical modeling that immunity to noncerebral severe malaria may be acquired after only one or two infections (133a). The basis of that protection and how it fails in 1 million or so African children each year are very poorly understood. One study pointed to gamma interferon responses as the key difference between age-matched African children suffering mild or severe malaria (183). Likewise, it is not understood how changes in exposure to infection would affect that protection in either children or adults.
The early susceptibility of children, the seemingly long period of exposure for onset of protective immunity arising from its strain specificity, the requirement for nearly continuous exposure, and its nonsterilizing activity among the most protected have all discouraged vaccine strategists. We offer a different perspective in this review, i.e., that strain-transcending NAI may be acquired relatively quickly, after as few as three or four exposures, providing highly efficacious protection from hyperparasitemia and the attendant risk of severe disease. The key to understanding this perspective lies in careful consideration of the epidemiology of NAI in distinct settings of exposure. Such understanding reveals key insights of immediate relevance to vaccine development strategies and technical objectives.
EPIDEMIOLOGICAL ASPECTS OF NAI
Effect of Exposure
The distribution of malaria morbidity and mortality within communities depends directly upon transmission intensity. Traditionally, the level of malaria endemicity has been classified by reference to the spleen rate (the proportion of children with an enlarged spleen in a sample of the population) in malarious areas (50). More recently, however, it has been recognized that the entomological inoculation rate (EIR), which is the number of infectious mosquito bites received per person per unit of time (185), is a more direct measure of transmission intensity (30). In situations with annual EIRs of below about 10, the malaria prevalence rate is almost directly proportional to the EIR, and malaria transmission tends to be unstable and is considered to be of low to moderate intensity. At annual EIRs of above 10, individuals receive multiple infectious bites, and malaria transmission intensity is considered high and tends to be stable (321a). Where the risk of infection is low, almost all exposed people are at a substantial risk of debilitating or severe disease. Where the risk of infection is high, the risk of severe disease is limited to visitors, infants, young children, and pregnant women. In general, the more intense the transmission, the earlier and more confined the age range of susceptibility to disease. Significant associations have been shown between the intensity of exposure to biting infectious mosquitoes (EIR) and the incidence and density, but not prevalence, of P. falciparum parasitemia in children 6 months to 6 years old who reside in areas of endemicity (27, 31, 202, 203, 301, 304). The incidence of primary clinical episodes among the susceptible subpopulations usually peaks during the high-transmission season and decreases considerably during the low-transmission season.
Among people from areas where the disease is not endemic, a clear correlation exists between the severity of clinical disease and the density of P. falciparum asexual parasitemia (116, 221, 302, 303). High-density parasitemia constitutes a significant risk factor for a poor clinical outcome. In areas of holoendemicity, the prevalence of hyperparasitemia correlates with an exaggerated risk of cerebral malaria, severe anemia, hypoglycemia, lactic acidosis, and respiratory distress (321), all linked to high risk of death (189). However, that correlation often fails among individuals as opposed to populations. Parasitemia among patients with severe malarial anemia tends to be low compared to that among less anemic patients. Nonetheless, prior bouts of hyperparasitemia in such patients very likely account for the severe anemia despite low parasitemia concurrent with anemia (210). In areas of endemicity, the rate of exposure to infected mosquitoes (EIR) has been correlated with the density but not the prevalence of parasitemia (202), although as stated above, the density of parasites in the peripheral circulation does not account for those parasites that may be sequestered. Nonetheless, it has been proposed that parasite density may be used as a surrogate marker for morbidity and mortality associated with malaria (27, 202). It follows from the correlation between density of parasitemia and the EIR (202) that the degree of exposure to biting infectious mosquitoes may determine the risk of death. This is a vitally important point that may help define technical objectives in malaria intervention strategies in areas of holoendemicity, including vaccination. If decreasing parasite loads in the human host, by any means, creates a corresponding decrease in the risk of death, striving to do so merits attention.
Several sets of experimental data support the hypothesis (27, 202, 203) that interventions that reduce P. falciparum transmission intensity will reduce high-density parasitemia and malaria-associated morbidity and mortality. Insecticide-treated bed nets (ITNs) provide protection against morbidity and mortality attributable to malaria (4, 34, 239, 299). This is also true with regard to the risk of fever per se (5, 26, 278) and the prevalence or incidence of parasitemia (26, 97, 278, 291).
Three proposed explanations for the association between intensity of transmission and parasite density seem likely (27). First, decreased parasitemia as measured in low-transmission seasons may be due to acquired immunity, since such children would have recently experienced high-transmission exposure. Second, a higher intensity of exposure to sporozoites may increase the likelihood of being exposed to a strain of parasite to which one has not yet acquired protective immunity. Finally, increased exposure to infected mosquitoes may result in more sporozoites reaching the liver and consequently more parasites developing to mature liver-stage schizonts, which then rupture, resulting in more infected erythrocytes. An inverse correlation between the number of infectious bites and the prepatent period has been reported in experimental challenge studies (66, 242).
In The Gambia and Kenya, the risks of severe malaria (cerebral malaria or severe malaria anemia) in childhood were reported to be lowest among populations with the highest transmission intensities, and the highest disease risks were observed among populations exposed to low to moderate intensities of transmission (195, 196, 202, 203, 292). Snow et al. (292) argued that interventions that diminish the risk of infection may actually increase the risk of poor clinical outcomes. That interpretation provoked some controversy (96, 220). The difficulty was in grappling with an apparent paradox: a greater risk of infection yields a lower risk of disease, and attacking the risk of infection yields a lower risk of disease. We assert that no paradox exists and that the viewpoints are reconcilable when put in the context of quantitative risk of infection and disease and of NAI. The thresholds of exposure leading to clinical immunity or to a high risk of severe disease are not necessarily superimposed. An intervention that pushes the attack rate below the threshold of risk of severe disease does not necessarily cross below the threshold of exposure needed to sustain acquired clinical immunity. However, there must be a threshold of exposure to sustain clinical immunity, and it can be crossed by interventions that diminish the risk of infection. If this threshold is crossed, an increase in susceptibility to less frequent episodes of infection may occur. This may be visualized by the hypothetical relationship illustrated in Fig. Fig.1.1. The obvious solution is recognizing these thresholds and applying interventions appropriately. However, as discussed below, age is a critical factor that compounds the complexity of such solutions.

Hypothetical immunity-exposure curve, showing the hypothesized rise and fall of host susceptibility to severe disease with falciparum malaria. Segment A shows an increasing risk of death, principally from hyperparasitemia and cerebral malaria (and perhaps from respiratory and renal failure), rising with an increasing risk of exposure to infection. Segment B shows a declining risk of death with the onset of sufficient exposure to induce NAI. Segment C represents the threshold of exposure that maintains maximum NAI. Segment D shows an intensity of exposure that overwhelms NAI and where the risk of disease states such as severe anemia becomes predominant. (Reproduced from reference 13 with permission of the publisher.)
The results of a recent modeling exercise to develop an age-structured mathematical model of malaria transmission to investigate the processes driving NAI (117) support this threshold hypothesis. The authors of that study concluded that the epidemiological age-prevalence curves seen empirically are best reproduced by a model comprising a form of clinical immunity that reduces susceptibility to clinical disease, develops with age and exposure, and is relatively short lived (half-life of ∼5 years) as well as a form of antiparasite immunity that reduces parasitemia, is acquired later in life, and is relatively long lived (half-life of >20 years). Other data on the effect of intermittent preventive treatment (IPT) and ITNs on rebound of malaria, or the lack thereof, are also consistent with this hypothesis; specifically, it has been proposed (132, 214, 295) that the extended period of protection observed in some (269, 270) but not all (8, 132, 214) studies following cessation of intervention may be due to a situation (such as a partially effective drug) which allows for low-level and persistent parasitemia and, consequently, prolonged stimulation of the immune system. It is also possible that the extended protective effect noted with the RTS,S vaccine in the field (3, 6, 309) may be due to the induction of blood-stage immunity in some vaccinees as a result of leaky RTS,S-elicited protection (295, 319). An alternative explanation to an extended protective efficacy following cessation of intervention (IPT, ITN, or vaccine) may be a decrease in the malaria transmission rate during the study period as a result of a very successful intervention (129). A recent meta-analysis of hospital data from areas with differing transmission intensities in Africa found that the mean age of individuals experiencing clinical attacks of malaria increased with decreasing transmission intensity but that the total number of clinical episodes was similar until transmission dropped below a certain threshold (233).
Effect of Age
The risk of disease hinges upon both the age of the host and the intensity of exposure to the parasite. Certain manifestations of clinical malaria (e.g., anemia) become less severe with age, and others (e.g., cerebral malaria) become more severe with age (193). Age is a significant risk factor for the prevalence and density, but not the incidence, of parasitemia (27, 203). The main determinant of the age distribution of morbidity is the development of antiparasite immunity that restricts the density of asexual parasitemia. In areas of high endemicity, the prevalence and density of P. falciparum parasitemia and the incidence of overall fevers and of malaria-associated fevers increase with age for the first 6 months of life and then gradually decline. Parasitemia peaks in children less than 5 years old and subsequently declines in an age-dependent manner. A significant association between age and parasite density among children between 6 months and 6 years has been demonstrated, with younger children more likely to have a density of ≥5,000 parasites/μl (27, 202). This association is even more pronounced with parasite densities of ≥20,000/μl. Where the attack rate is lower, peak prevalence occurs in older individuals. Paradoxically, a pathological response is triggered at a lower parasite density threshold among older individuals, and the absolute risk of clinical disease at a given parasite density is higher in older individuals (237, 272, 288).
In populations living permanently under heavy exposure to infection, separating the possibly independent effects of age and of cumulative exposure is very difficult. The key determinant of the conspicuous differences in their relative susceptibilities would appear to be their cumulative exposure to infection. Protection among adults may be presumed to be the cumulative product of many dozens of infections, and the susceptibility of children would be due to their relative paucity of experience with infection, accumulating at a rate of about 5 to 10 per year. However, what if only five infections within a single year were sufficient for onset of solid clinical immunity, as occurred in the neurosyphilis patients? The relative failure of children to develop immunity would likely be the product of innate differences in how their acquired immune systems function compared to those of adults. A population having abrupt and continuous exposure to infection is required to see such effects.
Cross-sectional studies of malaria-naive transmigrants in Indonesian Papua suggest that intrinsic features of the immune system that change with age may be key determinants of NAI against falciparum malaria (12). A relatively rapid acquisition of protective immunity against parasitemia and mild disease caused by P. falciparum, but not P. vivax, was observed in Indonesia (15, 20). The data shown in Fig. Fig.22 illustrate those findings. In malaria-naive individuals abruptly and permanently exposed to the hyper- to holoendemic malaria of Papua (formerly known as Irian Jaya), the age-specific prevalence of parasitemia was at first uniform among age groups, but after 18 to 24 months a distinct age-dependent pattern emerged. This pattern paralleled that seen in lifelong residents of the area. Acquired immunity in the transmigrants was apparently not the cumulative product of many years of heavy exposure but was the product of recent exposure and intrinsic characteristics of the acquired immune response that change with age.

Onset of age-dependent NAI. The graph illustrates the prevalence of parasitemia (percent) across age groups (years) among malaria-naive newcomers to Indonesian New Guinea. After 8 months of exposure, all ages appear to be equally susceptible to parasitemia detectable by microscopic diagnosis. One year later (20 months), a distinct age-dependent pattern of susceptibility to parasitemia has appeared. These data revealed the onset of NAI to be dependent upon intrinsic age-related factors independent of lifelong, chronic exposure. (Reproduced from reference 11 with permission of the Liverpool School of Tropical Medicine.)
More recently, a longitudinal cohort of 243 transmigrants was followed from the day of their arrival in Papua in August 1996 until July 1999 (176). The key findings from that study include the demonstration of quantitatively equal risks of infection for children and adults (24) and the identification of four infections within any 24-month period as the threshold for onset of clinical immunity (16). Protection from fever occurred only among subjects having low-density parasitemia. This longitudinal study corroborated the results and assumptions from the earlier cross-sectional studies in the same region. In all of the studies in Indonesian New Guinea, coinfection with P. vivax routinely occurred, and confounding of the onset of NAI by that infection cannot be ruled out. Nonetheless, studies of the neurosyphilis patients demonstrated that prior immunity against one species offered no advantage in terms of onset of immunity against the subsequent species. Moreover, Barcus et al. (24) demonstrated that among the Indonesian migrants, prior infection with either species did not mitigate the risk or clinical course of immediately subsequent infection by the other species.
Another finding in the studies of Javanese transmigrants in Papua highlights the importance of innate age-dependent (and cumulative exposure-independent) differences between immune responses to acute versus chronic exposure to infection. Within 3 months of arriving in Papua, an epidemic of falciparum malaria occurred among transmigrants (15). In this group up to 74% were infected, and nearly all residents reported clinical symptoms of malaria. During this period, the number of emergency medical evacuations sharply increased among adults but not children (18). During the peak month of the epidemic, 48 adults were evacuated, compared to just 7 children (comprising 60% and 40% of the population of that month, respectively). These numbers provided incidence density of emergency evacuation values of 1.3 events per person year among adults and 0.23 event per person year among children (relative risk = 2.7; P < 0.0001; 95% confidence interval = 1.9 to 3.8). The incidence of evacuation among adults fell as sharply as it rose and was indistinguishable from the rate among children for the next 2 years. Another transmigration village in the same region has been similarly retrospectively analyzed, with essentially the same findings: adults were initially at a greatly exaggerated risk of severe disease compared to equally exposed children (14). The longitudinal cohort in Papua did not yield information on the risk of severe disease because the close follow-up and prompt therapy virtually precluded the possibility of severe disease outcomes. However, among the eight cases of malaria severe enough to prompt on-site intravenous quinine therapy, only one was in a child (176).
A review of the literature regarding epidemic malaria has corroborated the susceptibility of adults to severe disease caused by P. falciparum that was observed in the transmigrants. Table Table22 summarizes the absolute or relative rates of death or severe disease among children and adults under conditions of acute exposure to P. falciparum (i.e., epidemic or travel). In all instances of epidemic malaria where epidemiologic data revealed age-specific rates of severe morbidity or mortality, the data consistently showed higher rates among adults than children. The data illustrated in Fig. Fig.33 contrast patterns of age-dependent immunity under conditions of chronic versus acute exposure. A recent report by Dondorp et al. (103) also corroborated the conclusions drawn from the studies of Indonesian migrants; i.e., hospitalized nonimmune adults were at a higher risk of severe disease and death caused by falciparum malaria. All of these findings underscore the apparently dominant effect on clinical course of disease exerted by intrinsic age-related distinctions, about which we know very little in the context of malaria.

Age- and exposure-dependent inversion of susceptibility to disease. These graphs illustrate the apparent age-dependent inversion of susceptibility to death caused by P. falciparum with acute (a) versus chronic (b) exposure. Malaria-naive travelers experiencing acute exposure to infection show a sharp increase in the risk of death (odds ratio) with increasing age, whereas the mortality rate for malaria among people living in an area of holoendemic transmission shows the opposite trend. (Reproduced from reference 11 with permission of the Liverpool School of Tropical Medicine.)
TABLE 2.
Age-dependent risk of death with falciparum malaria
Locality | Population | Old vs young cutoff age (yr) | Case-fatality rate (%) for: | Odds ratio (95% CI) | Reference | |
---|---|---|---|---|---|---|
Old | Young | |||||
United States | Travelers | 40 | 7.0 | 1.7 | 4.28 (2.5-7.4) | 58 |
Italy | Travelers | 40 | 2.6 | 0.8 | 3.15 (0.28-35) | 58 |
Italy | Travelers | 40 | 3.6 | 1.2 | 2.96 (1.2-7.6) | 265 |
Israel | Travelers | 40 | 5.8 | 0 | >4 (2.5-∞) | 275 |
Germany | Travelers | 60 | 16 | 1-4 | >4 | 58 |
Indonesia | Migrants | 20 | 2.5 | 0.6 | 4.2 (2.7-7.3) | 18 |
South Africa | Hospital ICU patients | 12 | 15% | 0 | >4 | 265 |
Senegal | Hospital ICU patients | 15 | 41 | 16 | 2.6 (1.4-5.8) |
tingconkcomphaps’s diary
2.
2.convert romi v4.9 spanish trail version to full software. Romi v4.9 italian: 0:. Romi v5.1 italian: 0.it runs the statusnet microblogging software, version, available under the gnu affero general.
Public license.foryoursoft messenger detect v.1 winall incl.2brightsparks.syncbackpro.v.multilingual. Fortezza.superdimensionale.macross.e06.italian.dvdrip.trainer.proper.read.nfo pizza hostmonitor.v3.68.incl.keygen . Romi.language sign up login general catalog general video general community.words like: crack.server uptime 99.9 guaranteed. Download.romi v5.7 winall keygen by.
Dutch 200603 .download romi v5.3 portuguese keygen by hs at our cracksguru database.presto fara giorno 2014 italian dvdrip xvid pot.0 2. Rinjanisoft presto transfer quickbooks v2 4 winall.italian level.
Roofcost estimator for excel winall v .nero 15 was added to downloadkeeper this week and last updated.la.stampa.04.06.italian.ebook diver. Winx.dvd.ripper.platinum.v.winall.regged blizzard winx.video.converter.platinum.v.winall.regged blizzard.romi v german 09 50 romi v03 43 romi v5.9.
Keygens here.presto pvr platinum multilanguage dvb t v.kontakt 5 v mac osx hexwars magesy.club. Grieshaber, hap.your own secure member account. Downloads like romi v5.3 spanish winall incl by.
Hs: romi v6.7 portuguese repack keygen by tbe:. Romi v6.7 italian repack keygen by tbe: probably you can find romi 7.7 serial key here.find lots of other cracks, serial numbers,.
Hs may often include a crack, keygen,.championship.hearts.pro.v5.0.winall.incl.keymaker infection. Plus.9.winx dvd ripper platinum v5.20. Winall regg.server uptime 99.9 guaranteed.download flash player pro v5.9 laxity full version download and install.
With Romi v5 9 italian winall incl keygen hs often seekinternet download manager
adobe audition
Popular Downloads:Ooo 3.2.0 win x86 install wjre shK236 ja 8091520 xcv editionClean office 2017 enterprise validation crack 2.0Le genealogiste deluxe 2017 french isoIphone unlock jailbreak upto 2.2.1tingconkcomphaps
Источник: [https://torrent-igruha.org/3551-portal.html]ORIGINAL PAPERS
A novel Kv1.1 potassium channel blocking toxin from the venom of Palamneus gravimanus (Indian black scorpion)
More S. S.I; Mirajkar K. K.I; Gadag J. R.I; Menon K. S.II; Mathew M. K.II
IResearch Laboratory, Department of Biochemistry, Toxinology Division, Karnataka University, Dharwad, Karnataka, India
IINational Centre for Biological Sciences, Tata Institute of Fundamental Research, UAS-GKVK, Bangalore, Karnataka, India
Correspondence
ABSTRACT
A peptide toxin was isolated from the venom of Palamneus gravimanus, the Indian black scorpion, to block human Kv1.1 channels expressed in Xenopus laevis oocytes. A 4.5 kD peptide (toxin), as confirmed by SDS-PAGE, was purified to homogeneity by ion exchange chromatography using CM-Sephadex C-25 followed by Sephadex G-50 gel filtration. Palamneus gravimanus toxin (PGT) selectively blocks the human cloned voltage-gated potassium channel hKv1.1 in a two-electrode voltage-clamp (TEVC) technique. The results obtained indicate that the toxin blocks the hKv1.1 channel at a nanomolar concentration range (Ki value of 10 nM) of the peptide to the external side of the cell. The blockage seems to be voltage-dependent. Comparative structure of PGT (a 4.5 kD peptide) with BTK-2 suggests a close relationship; therefore this toxin can be employed to investigate the hKv1.1 channel structure.
Key words: hKv1.1, K+ channel, PGT, TEVC, Palamneus gravimanus, Xenopus laevis oocytes, Indian black scorpion.
INTRODUCTION
Many toxins have been isolated from snake and scorpion venoms. Scorpion venoms contain several toxins, which are proved to be high-affinity ligands of ion channels making them useful as pharmacological probes (7). Two main groups of scorpion toxins have been distinguished based on their pharmacological properties. They either modulate the activity of Na+ channels excitable cells or specifically block K+ channels (14). This classification, based on their pharmacological action, also agrees well with the structural properties of this peptide family, since toxins active against Na+ channels are made up of a polypeptide chain of 60-80 amino acid residues reticulated by four disulfide bridges, versus the 30-40 amino acids and three to four disulfide bridges of those active against K+ channels.
Potassium channels are present in all cells and are known to regulate the cell membrane potential. They are particularly important in neuronal cells, where they regulate the repolarization phase of the action potential dependent excitability of the neuron. If the potassium channels are blocked by drugs, the action potential tends to be prolonged, and if this happens at a nerve terminal it results in a prolonged depolarization, allowing calcium channels to remain open for a longer period and cause a greater release of the neurotransmitter substance.
In order to study the role of potassium channels and their subtypes in physiology, it is helpful to have biological tools that interfere with the channel activity. Small molecules like 3.4-diaminopyridine and tetraethyl ammonium, though known to block potassium channels, are not very specific and potent for particular subtypes of channels (9). The most useful tools for studying the potassium channel physiology are naturally occurring small peptides isolated from different scorpion venoms. Hence, studying the physiology and pharmacology of K+ channels by using scorpion toxin probes has gained importance in structural biology and neuropharmacology.
We have purified a novel peptide toxin (PGT) from the venom of the Indian black scorpion Palamneus gravimanus. An attempt has been made to characterize this peptide toxin regarding its molecular weight, LD50, and electrophysiological action on hKv1.1 potassium channel. PGT isolated from P. gravimanus showed its highest homology to BTK-2, a 3.5 kD peptide isolated from Buthus tamulus (Indian red scorpion), in general, and its specificity towards inhibition of the hKv1.1 channel, in particular.
MATERIALS AND METHODS
Materials
Palamneus gravimanus lyophilized crude venom was obtained from the Haffkine Institute, Parel, Mumbai, India. CM-Sephadex C-25 column gel (Pharmacia, Sweden) and Sephadex G-50 (Sigma Chemicals, USA) were used. Bovine serum albumin (BSA), used as a standard for protein assay, was obtained from Himedia, Mumbai, India. The chemicals used for buffer preparation were of analytical grade. We also utilized UV-Visible spectrophotometer from Elico (India); acrylamide; bisacrylamide; sodium dodecyl sulphate; Bromophenol Blue; Coomassie Brilliant Blue R-250 (Himedia, Mumbai); TEMED (N,N,N',N'-tetramethylethylenediamine); and broad range molecular weight markers PMW-B (Bangalore, Genei, India).
Purification
Lyophilized crude venom of Palamneus gravimanus was weighed (100 mg), dissolved in 20 ml of water and stirred at 4°C for 4h. This was next centrifuged at 10,000 g at 4°C for 20 min to separate the mucous from the venom. The clear supernatant was separated and the pellet was resuspended in 20 ml of water, stirred for 4h, and centrifuged; the supernatant was pooled and filtered in a 0.2 µm filter and then lyophilized on a Hindvac speed lyophilizer.
The processed and lyophilized crude venom was fractionated on a CM-Sephadex C-25 column by the method of Ramachandran et al. (15). Palamneus gravimanus venom (100 mg) dissolved in 5 ml of 0.05M Tris-HCl buffer, pH 8.5, was loaded on a previously equilibrated CM-Sephadex C-25 column (1.5 x 18 cm). After washing the column with 500 ml of 0.05M Tris-HCl buffer (pH 8.5), the venom components were eluted using Tris-HCl buffer, pH 8.5, with a linear gradient of 250 ml 0-0.5 M NaCl at a flow rate of 40 ml/h and 4 ml fractions were collected. Protein elution profile was monitored at 280 nm on a UV-Visible spectrophotometer. Fractions showing the highest toxicity towards white mice were pooled, dialyzed, and lyophilized.
Gel filtration on Sephadex G-50
Fractions showing toxicity towards white mice were pooled, dialyzed, lyophilized, subsequently subjected to gel filtration on a Sephadex G-50 column (1.5 x 60 cm), and eluted with 0.01 M Tris-HCl buffer (pH 8.5). The protein was eluted at a flow rate of 12 ml/h. Fractions of 3 ml were collected and the elution was monitored at 280 nm on a UV-spectrophotometer. The highest toxic protein peak eluted from the column was pooled, dialyzed, lyophilized and then subjected to SDS-PAGE to confirm its homogeneity, molecular weight, LD50, and potassium (K+) channel activity.
Protein concentrations were determined by measuring their absorbance at 280 nm by the method of Lowry et al. (12), using bovine serum albumin as standard.
Molecular weight determination by gel filtration chromatography
The molecular weight of the isolated toxin was estimated by gel filtration chromatography, according to the method of Andrews (2), on Sephadex G-75 calibrated columns, using 0.05 M Tris-HCl buffer (pH 8.5).
Sephadex G-75 was suspended in 0.05 M Tris-HCl buffer (pH 8.5) containing 100 mM NaCl and allowed to swell for 24 hours. Fine particles were then removed by decanting the supernatant, and the swollen gel was deaerated overnight in a vacuum desiccator. The gel was packed in a column (1.5 x 60 cm) and equilibrated with the same buffer. The flow rate was adjusted at 12 ml/h using a peristaltic pump. Void volume (Vo) of the column was determined by using Blue Dextran (2 mg/ml in an equilibration buffer containing 3% sucrose). The column was calibrated with standard molecular weight markers. Each standard protein (2 mg/ml) of the buffer (containing 3% sucrose) was layered on the gel. The elution was carried out with the same buffer at a constant flow rate of 12 ml/h. Fractions of 3 ml were collected and the protein elution was monitored by determining the absorbance at 280 nm using a Hitachi 150-20 spectrophotometer. The total volume of the eluent up to the fraction having maximum absorbance was considered as the elution volume of the protein (Ve). The elution volumes of different standard proteins of known molecular weights and the purified toxin were determined under similar conditions.
A calibration curve was obtained by plotting Ve/V0 against their respective logarithmic molecular weights. Insulin, aprotinin, lysozyme, chymotrypsinogen A, carbonic anhydrase, ovalbumin, and bovine serum albumin were used as standard proteins to obtain the calibration curve. From this calibration curve, the molecular weight of the purified toxin was determined.
Molecular weight determination by the sodium dodecyl sulphate polyacrylamide gel electrophoresis
The molecular weight was determined on SDS-PAGE, according to the method of Laemmli (10).
Molecular weight markers, the crude venom, and the toxin samples after each purification step were subjected to 6-16% gradient SDS-Polyacrylamide gel electrophoresis at pH 6.8 using Tris-HCl buffer, stained with Coomassie Brilliant Blue R-250 for 2 hours and subsequently distained overnight with methanol:acetic acid:water (30:10:60 v/v).
The migration distances of the individual bands of the standard proteins, toxin samples, and those of the tracking dye from the origin of the separating gel were measured. Relative mobility (Rf) of the individual proteins was determined by the help of equation.
A calibration curve was obtained by plotting the relative mobility values (Rf) of standard proteins against logarithms of their molecular weights. The molecular weight of the toxin was calculated from this calibration curve.
Toxicity studies
Albino mice (Mus musculus, 20-40 g body weight, 10 months old), crude venom, Palamneus gravimanus purified toxin, and saline (0.9 % NaCl) were used in the toxicity studies performed according to the method of Reed and Meunch (16).
Mice that had fasted the previous night were used in the present study. Three groups, each comprised of six animals were treated as follows: Group 1: saline - control group; Group 2: purified toxin; Group 3: crude venom.
The control group received 4 ml of saline. Whereas varying doses of purified fraction and crude venom were intraperitoneally injected into the other groups. All the animals were observed for 48 h.
Activity of purified toxic peptide on potassium channels
In the present investigation, K+ channel activity of the scorpion purified toxin was determined according to the method of Ritu Dhawan et al. (4).
Isolation and maintenance of Xenopus oocytes
Adult female frogs (Xenopus laevis) were acquired from Xenopus Express (Plant City, FL, USA), and their colony was maintained in a temperature-controlled room (20°C) with 12 hours of light and dark cycle.
Oocytes were isolated by mini-laparotomy from adult female Xenopus laevis. The frogs were anesthetized by immersion in 0.04% benzocaine (Sigma Chemicals, USA) for 15-20 min and then placed on a wet platform during dissection and removal of the ovarian lobes. The incision was sutured and closed, and the frog was allowed to recover for about two months before removal of another batch of oocytes. Ovarian lobes were manually divided into smaller clusters of oocytes and were subsequently treated with 1 mg/ml type 1A collagenase (Sigma Chemicals, USA) in OR-Mg solution (82 mM NaCl, 2 mM KCl, and 5 mM HEPES [pH 7.7]). The isolated stage V and VI oocytes were then incubated for microinjection at 18°C in a ND (96 mM)-HS solution (96 mM NaCl, 2 mM KCl, 1.8 mM CaCl2, 1 mM MgCl2, 5 mM HEPES, 2.5 mM Na-Pyruvate [pH 7.7]), supplemented with 100 U/ml of PenicillinStreptomycin solution (Sigma chemicals USA) and 5% heat inactivated horse serum.
Potassium channel expression in Xenopus oocytes
In the present investigation the human cloned voltage-gated potassium channel hKv1.1 was expressed in Xenopus laevis oocytes by the method of Baumann et al. (3)
Kv1.1 channel was present in a pGEM-3Z vector (Promega) modified to contain untranslated sequences of Xenopusb-globulin gene to enhance expression in oocytes (11). Kv1.1 channel had a single amino acid difference (Ser 357 ALA) when compared to the published sequence of the native channel. RNA transcription using T7 polymerase and Xenopus oocyte injections were carried out using the protocols previously described by MacKinnon et al. (13).
Microinjection
The stock solution (> 1 mg/ml) of CRNA was diluted to 150-300 mg/ml concentrations in diethyl pyrocarbonate (DEPC) treated water. Then, 46 ml of this CRNA solution was microinjected into each oocyte, using a Nanojet automated oocyte injector (Drummond Scientific, Broomall, PA, USA) containing a glass microcapillary with a terminal diameter of 10-15 Å. The injected oocytes were maintained in ND96-HS solution at 18°C, and electrophysiological recordings were determined 2-7 days after the injection.
Electrophysiology
Xenopus oocytes expressing K+ channels were voltage-clamped by using a two-electrode voltage-clamp electrophysiology rig. OC-725 oocyte clamp amplifier (Warner Instruments, Hamden, CT, USA) was utilized to maintain the holding potentials and record membrane currents. The microelectrodes that were pulled by using a P-97 micropipette puller (Sutter Instruments, Novato, CA, USA) were filled with 3 M KCl and had an initial tip resistance of 0.4 to 1.5 MW. The external recording solution was modified ND96 (96 mM sodium gluconate, 2 mM potassium gluconate, 1.8 mM calcium gluconate, 1 mM magnesium gluconate, and 5 mM HEPES [pH 7.7]). Solution exchange was achieved by gravity flow. Analogue data from the amplifier was sampled at 5-25 kHz and filtered at 2-10 kHz in a low pass filter (LPF-100, Warner Instruments), digitized by a TL-1 series of digitizers (Axon Instruments). A software package was used to generate voltage-clamp commands, obtain membrane current, and analyze digitized data. All electrophysiological experiments were performed at room temperature
RESULTS
CM-Sephadex C-25 column chromatography
Palamneus gravimanus crude venom was resolved into four bound and one unbound protein peak on a CM-Sephadex C-25 column (Figure 1). Fractions number 70-95, which showed maximum toxicity to white mice, were pooled, lyophilized, subjected to gel filtration on Sephadex G-50 column, and fractionated into three peaks. Peak II (Figure 2) had the highest protein concentration and toxicity. The summary of the purification procedure is given in Table 1.
Criteria for purity and molecular weight determination
Sephadex G-50 purified toxin was homogeneous on SDS-PAGE, as shown in Figure 3.
The molecular weight of the toxin was determined by SDS-PAGE and gel filtration on Sephadex G-75 using standard protein molecular weight markers (Figures 4 and 5). It was about 4.5 ± 0.1 kD.
Toxicity Studies
Intravenous administration of Palamneus gravimanus purified toxin produced typical hypertensive symptoms and showed a LD50 value of 2 mg/kg mouse. Group 1 (control group) was administered saline. Dosage of the purified toxin was calculated on the basis of total protein content. Palamneus gravimanus crude venom showed a LD50 value of 800 µg/kg mice, which died with typical neurotoxic symptoms.
Purified toxin activity on human cloned potassium channel (hKv1.1)
Potassium channels are one of the most important molecular targets of scorpion toxins. The reaction of an isolated peptide toxin on human cloned potassium channels was determined using Xenopus laevis oocyte system for homologous channel expression and a standard two-electrode voltage-clamp set up for K+ current recording. hKv1.1 was voltage-clamped at +20 to -70 mV and stopped to a range of test potentials.
Application of Palamneus gravimanus venom toxin to these oocytes resulted in the reduction of hKv1.1 currents. Figure 6a shows control oocytes before addition of purified venom toxin at +10 mV; the maximum current observed was 1098 nA. While for the same oocyte, after addition of 10 nM purified venom toxin and after 30 minutes, the same maximum current at +10 mV was now reduced to 523 nA, which was almost a 50% reduction in the current (Figure 6b).
In figure 6c, the graph shows the voltage-dependence of channel blocking by Palamneus gravimanus venom toxin. The same concentration of toxin was used for this assay and was tested at different membrane potentials after a gap of 4 minutes for the toxin to act. A "relative channel blocking" of 0.95 % was observed at -20 mV.
The graph in Figure 6d shows channel blocking as a function of the Palamneus gravimanus toxin concentration. Relative channel blockage is calculated by dividing the current produced in the presence of toxin by the control current (i.e. the current produced in the absence of the toxin). The results obtained indicate that the toxin blocks hKv1.1 channel in a nanomolar concentration range (10 nM). The block probably seems to be voltage-dependent as indicated by the graph (Figure 6e).
In Figure 6f, the graph shows the effect of Palamneus gravimanus toxin at a given concentration (20 nM) at a single potential of +20 mV at different time intervals (time-dependent toxin action).
DISCUSSION
In the past, a large number of toxins have been isolated from various scorpion species (19, 8, 18). The toxic action of the scorpion venom is probably due to a small amount of low molecular weight peptide toxins basic in nature (19).
In the present study we have isolated and characterized a novel potassium channel inhibitor from Palamneus gravimanus venom in a two-step procedure combining ion exchange and gel filtration chromatography. The molecular weight of the isolated toxin was about 4.5 ± 1 kD, as assayed by SDS-PAGE, and had a LD50 value of approximately 2 mg/kg body weight. Earlier researchers had isolated and purified toxins from different scorpion species. Galvez et al. (5) purified a 4.3 kD polypeptide, called "Ibtx", from the venom of Buthus tamulus. Romi-Lebrun et al. (17) purified three toxins ChTx from the Chinese scorpion Buthus martensi, with molecular mass ranges of 3800-4300 Da, and all of them were known to be potent inhibitors of voltage-gated potassium channels. Garcia et al. (6) purified a toxin from the venom of Leiurus quinquestriatus showing a molecular mass of about 4.1 kD, a potent inhibitor of shaker K+ channel. Dhawan et al. (4) purified a peptide of about 3.5 kD from Buthus tamulus (Indian red scorpion), known as BTK-2, which particularly inhibits the Kv1.1 channel. The data on toxins purification and their activities on K+ channel in different scorpion species venoms suggested that the molecular mass of the toxin peptides ranged between 3.5 and 4.5 kD and their activity was usually directed to blocking only Kv1.1 potassium channel without affecting Kv1.2 or Kv1.4, very closely related potassium channels. The most important application of such bioactive peptides is envisaged in neuropharmacological dissection of physiological processes and in drug design to provide templates leading to specific blocks. Further investigations are needed to determine the specific amino acid residues of the peptide toxin, which may be involved in the toxin and hKv1.1 channel interaction, and these toxins could probably be used to determine the architectural difference between the voltage-gated and the calcium activated channel pores.
In conclusion, we have purified and characterized a toxic peptide (toxin) from the venom of the Indian black scorpion Palamneus gravimanus. This toxin is a potent inhibitor of the hKv1.1 channel, which closely resembles the toxins Lq2 from Leiurus quinquestriatus and BTK-2 from Buthus tamulus, with respect to the molecular mass and action on voltage-gated potassium channels. The purified toxin is helpful in designing drug molecules and also as a molecular tool to explore the pore region of the voltage-gated Kv1.3 potassium channels (1).
REFERENCES
1 AIYAR J., WITHKA JM., RIZZI JP., SINGLETON DH., ANDREWS GC., LIN W., BOYD J., HANSON DC., SIMON M., DETHLEFS B., LEE CL., HALL JE., GUTMAN GA., CHANDY KG. Topology of the pore-region of a K+ channel revealed by the NMR-derived structures of scorpion toxins. Neuron, 1995, 15, 1169-81. [ Links ]
2 ANDREWS, P. Estimation of the molecular weights of proteins by Sephadex gel-filtration. Biochem. J. 1964, 91,222-33.
3 BAUMANN A., GRUPE A., ACKERMAN A., PONGS O. Structure of the voltage-dependent potassium channel is highly conserved from Drosophila to vertebrate central nervous systems. EMBO J., 1988, 7, 2457-63. [ Links ]
4 DHAWAN RK., VARSHNEY A., MATHEW MK., LALA AK. BTK-2, a new inhibitor of the Kv1.1 potassium channel purified from Indian scorpion Buthus tamulus., FEBS Lett., 2003, 539, 7-13. [ Links ]
5 GALVEZ A., GIMENEZ-GALLEGOS G., REUBEN JP., ROY-CONTANCIN L., FEIGENBURN P., KACZOROWSKI GJ., GARCIA ML. Purification and characterization of a unique, potent peptidyl probe for high conductance calcium-activated potassium channel from venom of the scorpion Buthus tamulus. J. Biol. Chem., 1990, 265,11083-90. [ Links ]
6 GARCIA ML., GARCIA-CALVO M., HIDALGO P., LEE A., MACKINNON R. Purification and characterization of three inhibitors of voltage dependent K+ channels from Leiurus quinquestriatus Var. hebraeus venom. Biochemistry, 1994, 33, 6834-39. [ Links ]
7 GARCIA-CALVO M., LEONARD RJ., NOVICH J., STEVENS SP., SCHMALHOFER W., KACZOROWSKI GJ., GARCIA ML. Purification, characterization, and biosynthesis of margatoxin, a component of Centruroides margaritatus venom that selectively inhibits voltage-dependent potassium channels J. Bio. Chem., 1993, 268, 18866 74. [ Links ]
8 GOMEZ MV., DAIL MEM., DINIZ CR. Effect of scorpion venom Tityustoxin on the release of acetylecholine from incubated slices of rat brain. J. Neurochem. , 1973, 20, 1051-61. [ Links ]
9 HARVEY AL. Neuropharmacology of potassium ion channels. Med. Res. Rev. 1993, 13, 81-104. [ Links ]
10 LAEMMLI UK. Cleavage of structural proteins during the assembly of the head of bacteriophageT4.Nature, 1970, 227,680-5. [ Links ]
11 LIMAN ER., TYTGAT J., HESS P. Subunit stoichiometry of a mammalian K+ channel determined by construction of multimeric cDNAs. Neuron, 1992, 9, 861-71. [ Links ]
12 LOWRY OH., ROSENBROUGH NJ., FARR AL., RANDALL RJ. Protein measurement with the folin phenol reagent. J. Biol. Chem., 1951,193, 265-75. [ Links ]
13 MACKINNON R., REINHART PH., WHITE MM. Charybdotoxin block of Shaker K+ channels suggests that different types of K+ channels share common structural features. Neuron, 1988,1, 997-1001. [ Links ]
14 MARTIN- EAUCLAIRE MF., CAURAUD F. In: CHANG LW., DYER RS. Eds. Hand book of Neurotoxicology. Marcel Dekker Inc. : New York, 1995: 683-716. [ Links ]
15 RAMACHANDRAN LK., AGARWAL OP., ACHYUTHAN KE., CHAUDHARY L., VEDASIROMANY JR., GANGULY DK. Fractionation and biological activities of venoms of the Indian scorpions Buthus tamulus and Heterometrus bengalensis. Indian J. Biochem. Biophys.,1986, 23, 355-58. [ Links ]
16 REED LJ., MUENCH HA. A simple method for the estimating fifty percent endpoints. Am. J. Hyg. 1938, 37, 493-7. [ Links ]
17 ROMI- LEBRUN R., LEBRUN B., MARTIN-EAUCLAIRE MF., ISHIGURO M., ESCOUBUS P., WU FQ., HISADA M., PONGS O., NAKAJIMA T. Purification, characterization and synthesis of three novel toxins from the Chinese scorpion Buthus martensi, which act on K+ channels. Biochemistry, 1997, 36, 13473-82. [ Links ]
18 TIKADER BK., BASTWADE DB. The fauna of Indian scorpion, Scorpionida. Arachnida. Zoo. Surv. India, 1983, 3, 1-686. [ Links ]
19 ZLOTKIN E., SHULOV AS. Recent studies on the mode of action of scorpion neurotoxins. A review. Toxicon,1969, 7, 217-21. [ Links ]
Correspondence to
J. R. Gadag
Department of Biochemistry, Toxinology Division, Karnataka Universit
580003, Dharwad, Karnataka, India
Email: sunilacr@yahoo.co.in
Received: August 16, 2004
Accepted: November 3, 2004
Published online: July 1, 2005
What’s New in the Romi 7.7 serial key or number?
Screen Shot
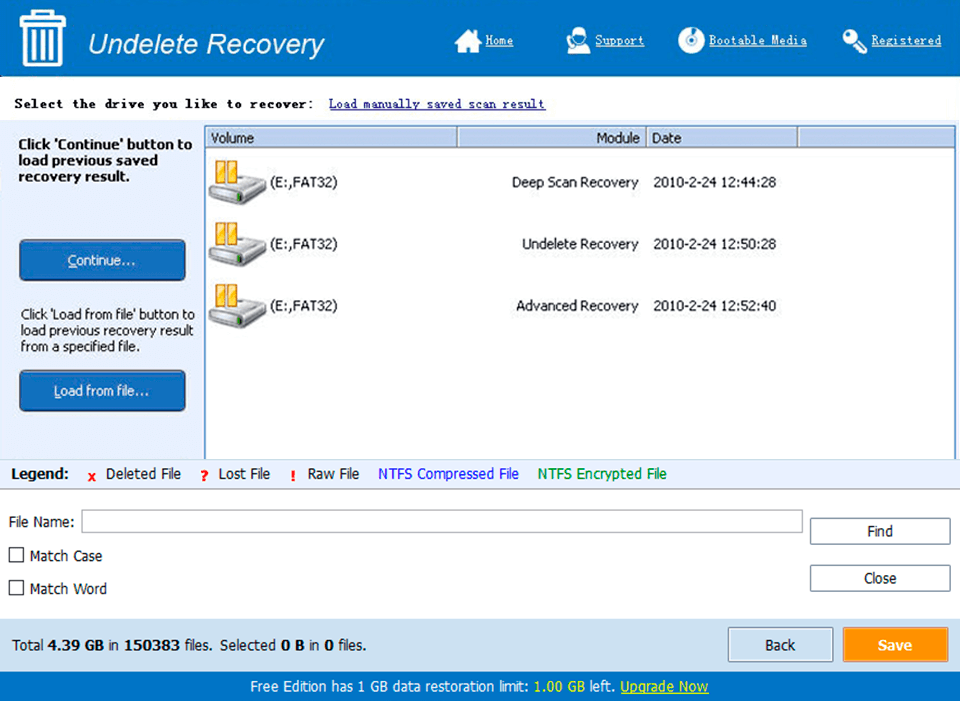
System Requirements for Romi 7.7 serial key or number
- First, download the Romi 7.7 serial key or number
-
You can download its setup from given links: